Diverse and unified mechanisms of transcription initiation in bacteria
Transcription of DNA is a fundamental process in all cellular organisms. The enzyme responsible for transcription, RNA polymerase, is conserved in general architecture and catalytic function across the three domains of life. Diverse mechanisms are used among and within the different branches to regulate transcription initiation. Mechanistic studies of transcription initiation in bacteria are especially amenable because the promoter recognition and melting steps are much less complicated than in eukaryotes or archaea. Also, bacteria have critical roles in human health as pathogens and commensals, and the bacterial RNA polymerase is a proven target for antibiotics. Recent biophysical studies of RNA polymerases and their inhibition, as well as transcription initiation and transcription factors, have detailed the mechanisms of transcription initiation in phylogenetically diverse bacteria, inspiring this Review to examine unifying and diverse themes in this process.
This is a preview of subscription content, access via your institution
Access options
Access Nature and 54 other Nature Portfolio journals
Get Nature+, our best-value online-access subscription
cancel any time
Subscribe to this journal
Receive 12 print issues and online access
206,07 € per year
only 17,17 € per issue
Buy this article
- Purchase on SpringerLink
- Instant access to full article PDF
Prices may be subject to local taxes which are calculated during checkout
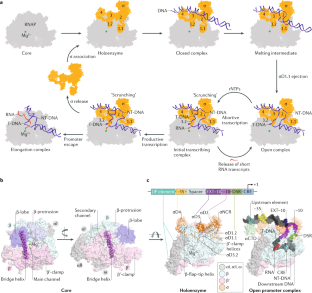
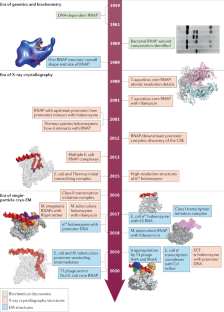
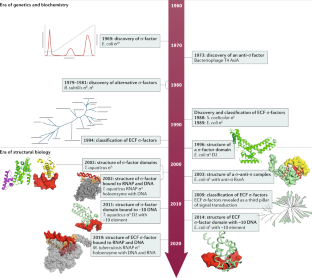
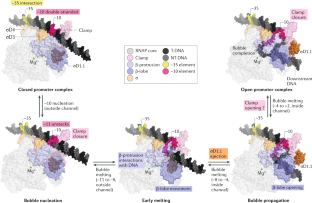
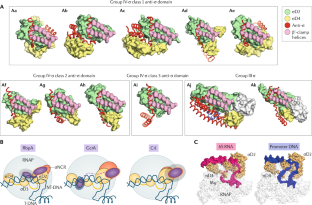

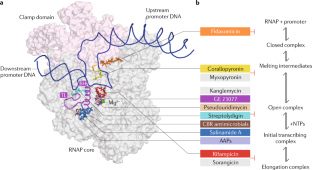
Similar content being viewed by others
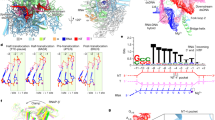
Structural basis for intrinsic transcription termination
Article 11 January 2023
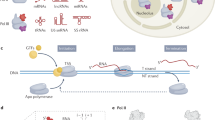
Structural insights into nuclear transcription by eukaryotic DNA-dependent RNA polymerases
Article 03 May 2022
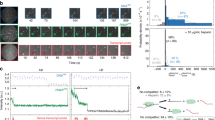
Alternative transcription cycle for bacterial RNA polymerase
Article Open access 23 January 2020
References
- Crick, F. Central dogma of molecular biology. Nature227, 561–563 (1970). CASPubMedGoogle Scholar
- Werner, F. & Grohmann, D. Evolution of multisubunit RNA polymerases in the three domains of life. Nat. Rev. Microbiol.9, 85–98 (2011). CASPubMedGoogle Scholar
- Decker, K. B. & Hinton, D. M. Transcription regulation at the core: similarities among bacterial, archaeal, and eukaryotic RNA polymerases. Annu. Rev. Microbiol.67, 113–139 (2013). CASPubMedGoogle Scholar
- Pribnow, D. Nucleotide sequence of an RNA polymerase binding site at an early T7 promoter. Proc. Natl Acad. Sci. USA72, 784–788 (1975). CASPubMedPubMed CentralGoogle Scholar
- Blombach, F., Smollett, K. L., Grohmann, D. & Werner, F. Molecular mechanisms of transcription initiation — structure, function, and evolution of TFE/TFIIE-like factors and open complex formation. J. Mol. Biol.428, 2592–2606 (2016). CASPubMedGoogle Scholar
- Cramer, P. Structure and function of RNA polymerase II. Adv. Protein Chem.67, 1–42 (2004). CASPubMedGoogle Scholar
- Burgess, R. R. Separation and characterization of the subunits of ribonucleic acid polymerase. J. Biol. Chem.244, 6168–6176 (1969). CASPubMedGoogle Scholar
- Zhang, G. et al. Crystal structure of Thermus aquaticus core RNA polymerase at 3.3 A resolution. Cell98, 811–824 (1999). First crystal structure of a DNA-dependent RNAP that provided high-resolution details of the core RNAP and defined many of the RNAP modules. CASPubMedGoogle Scholar
- Cramer, P. Architecture of RNA polymerase II and implications for the transcription mechanism. Science288, 640–649 (2000). CASPubMedGoogle Scholar
- Hirata, A., Klein, B. J. & Murakami, K. S. The X-ray crystal structure of RNA polymerase from Archaea. Nature451, 851–854 (2008). CASPubMedPubMed CentralGoogle Scholar
- Burgess, R. R., Travers, A. A., Dunn, J. J. & Bautz, E. K. F. Factor stimulating transcription by RNA polymerase. Nature221, 43–46 (1969). Demonstrated that a core RNAP required a σ-factor for transcription. CASPubMedGoogle Scholar
- Gruber, T. M. & Gross, C. A. Multiple sigma subunits and the partitioning of bacterial transcription space. Annu. Rev. Microbiol.57, 441–466 (2003). CASPubMedGoogle Scholar
- Feklistov, A., Sharon, B. D., Darst, S. A. & Gross, C. A. Bacterial sigma factors: a historical, structural, and genomic perspective. Annu. Rev. Microbiol.68, 357–376 (2014). CASPubMedGoogle Scholar
- Campbell, E. A. et al. Structure of the bacterial RNA polymerase promoter specificity sigma subunit. Mol. Cell9, 527–539 (2002). CASPubMedGoogle Scholar
- Callaci, S. & Heyduk, T. Conformation and DNA binding properties of a single-stranded DNA binding region of σ 70 subunit from Escherichia coli RNA polymerase are modulated by an interaction with the core enzyme. Biochemistry37, 3312–3320 (1998). CASPubMedGoogle Scholar
- Murakami, K. S., Masuda, S. & Darst, S. A. Structural basis of transcription initiation: RNA polymerase holoenzyme at 4 Å resolution. Science296, 1280–1284 (2002). CASPubMedGoogle Scholar
- Vassylyev, D. G. et al. Crystal structure of a bacterial RNA polymerase holoenzyme at 2.6 Å resolution. Nature417, 712–719 (2002). High-resolution structure of RNAP holoenzyme showing the housekeeping σ-factor domain architecture in context with RNAP. CASPubMedGoogle Scholar
- Danson, A. E., Jovanovic, M., Buck, M. & Zhang, X. Mechanisms of σ54-dependent transcription initiation and regulation. J. Mol. Biol.431, 3960–3974 (2019). CASPubMedPubMed CentralGoogle Scholar
- Hawley, D. K. & McClure, W. R. Compilation and analysis of Escherichia coli promoter DNA sequences. Nucleic Acids Res11, 2237–2255 (1983). CASPubMedPubMed CentralGoogle Scholar
- Simpson, R. B. The molecular topography of RNA polymerase-promoter interaction. Cell18, 277–285 (1979). CASPubMedGoogle Scholar
- Ross, W. et al. A third recognition element in bacterial promoters: DNA binding by the alpha subunit of RNA polymerase. Science262, 1407–1413 (1993). Describes the discovery of upstream elements. CASPubMedGoogle Scholar
- Barne, K. A., Bown, J. A., Busby, S. J. & Minchin, S. D. Region 2.5 of the Escherichia coli RNA polymerase sigma70 subunit is responsible for the recognition of the ‘extended-10’ motif at promoters. EMBO J.16, 4034–4040 (1997). CASPubMedPubMed CentralGoogle Scholar
- Haugen, S. P. et al. rRNA promoter regulation by nonoptimal binding of σ region 1.2: an additional recognition element for RNA polymerase. Cell125, 1069–1082 (2006). CASPubMedGoogle Scholar
- Zhang, Y. et al. Structural basis of transcription initiation. Science338, 1076–1080 (2012). CASPubMedPubMed CentralGoogle Scholar
- Feklistov, A. & Darst, S. A. Structural basis for promoter −10 element recognition by the bacterial RNA polymerase σ subunit. Cell147, 1257–1269 (2011). First high-resolution view describing the interaction of the σ-factor with the −10 element. CASPubMedPubMed CentralGoogle Scholar
- Hubin, E. A., Lilic, M., Darst, S. A. & Campbell, E. A. Structural insights into the mycobacteria transcription initiation complex from analysis of X-ray crystal structures. Nat. Commun.8, 16072 (2017). CASPubMedPubMed CentralGoogle Scholar
- Murakami, K. S. Structural basis of transcription initiation: an RNA polymerase holoenzyme-DNA complex. Science296, 1285–1290 (2002). CASPubMedGoogle Scholar
- Bae, B., Feklistov, A., Lass-Napiorkowska, A., Landick, R. & Darst, S. A. Structure of a bacterial RNA polymerase holoenzyme open promoter complex. eLife4, e08504 (2015). High-resolution crystal structure of a transcription initiation complex with a complete bubble and nascent RNA. PubMed CentralGoogle Scholar
- Zuo, Y. & Steitz, T. A. Crystal structures of the E. coli transcription initiation complexes with a complete bubble. Mol. Cell58, 534–540 (2015). First crystal structure at mid-range resolution of a transcription initiation complex with a complete bubble and nascent RNA. CASPubMedPubMed CentralGoogle Scholar
- Cortes, T. et al. Genome-wide mapping of transcriptional start sites defines an extensive leaderless transcriptome in mycobacterium tuberculosis. Cell Rep.5, 1121–1131 (2013). CASPubMedPubMed CentralGoogle Scholar
- Shell, S. S. et al. Leaderless transcripts and small proteins are common features of the mycobacterial translational landscape. PLoS Genet.11, e1005641 (2015). PubMedPubMed CentralGoogle Scholar
- Chakraborty, A. et al. Opening and closing of the bacterial RNA polymerase clamp. Science337, 591–595 (2012). Used single-molecule fluorescence energy transfer experiments to detect the motions of the RNAP clamp. CASPubMedPubMed CentralGoogle Scholar
- Duchi, D., Mazumder, A., Malinen, A. M., Ebright, R. H. & Kapanidis, A. N. The RNA polymerase clamp interconverts dynamically among three states and is stabilized in a partly closed state by ppGpp. Nucleic Acids Res.46, 7284–7295 (2018). CASPubMedPubMed CentralGoogle Scholar
- Feklistov, A. et al. RNA polymerase motions during promoter melting. Science356, 863–866 (2017). CASPubMedPubMed CentralGoogle Scholar
- Ruff, E. F. et al. E. coli RNA polymerase determinants of open complex lifetime and structure. J. Mol. Biol.427, 2435–2450 (2015). CASPubMedPubMed CentralGoogle Scholar
- Saecker, R. M., Record, M. T. & deHaseth, P. L. Mechanism of bacterial transcription initiation: RNA polymerase - promoter binding, isomerization to initiation-competent open complexes, and initiation of RNA synthesis. J. Mol. Biol.412, 754–771 (2011). CASPubMedPubMed CentralGoogle Scholar
- Buc, H. & McClure, W. R. Kinetics of open complex formation between Escherichia coli RNA polymerase and the lac UV5 promoter. Evidence for a sequential mechanism involving three steps. Biochemistry24, 2712–2723 (1985). Early evidence that transcription initiation occurs in multiple steps. CASPubMedGoogle Scholar
- Roe, J.-H., Burgess, R. R. & Record, M. T. Temperature dependence of the rate constants of the Escherichia coli RNA polymerase-λPR promoter interaction: assignment of the kinetic steps corresponding to protein conformational change and DNA opening. J. Mol. Biol.184, 441–453 (1985). Early kinetic probing of the transcription rate constants that set the stage for future kinetic studies of promoter melting by RNAP. CASPubMedGoogle Scholar
- Ruff, E. F., Record, M. T. & Artsimovitch, I. Initial events in bacterial transcription initiation. Biomolecules5, 1035–1062 (2015). CASPubMedPubMed CentralGoogle Scholar
- Hubin, E. A. et al. Structure and function of the mycobacterial transcription initiation complex with the essential regulator RbpA. eLife6, e22520 (2017). One of the first structures of mycobacterial RNAP with detailed quantitative analysis comparing the kinetics of open promoter formation by mycobacteria andE. coliRNAPs and the effects of transcription factors on this process. PubMedPubMed CentralGoogle Scholar
- Rammohan, J. et al. CarD stabilizes mycobacterial open complexes via a two-tiered kinetic mechanism. Nucleic Acids Res.43, 3272–3285 (2015). CASPubMedPubMed CentralGoogle Scholar
- Haugen, S. P., Ross, W. & Gourse, R. L. Advances in bacterial promoter recognition and its control by factors that do not bind DNA. Nat. Rev. Microbiol.6, 507–519 (2008). CASPubMedPubMed CentralGoogle Scholar
- Whipple, F. W. & Sonenshein, A. L. Mechanism of initiation of transcription by Bacillus subtilis RNA polymerase at several promoters. J. Mol. Biol.223, 399–414 (1992). CASPubMedGoogle Scholar
- Schroeder, L. A. & deHaseth, P. L. Mechanistic differences in promoter DNA melting by Thermus aquaticus and Escherichia coli RNA polymerases. J. Biol. Chem.280, 17422–17429 (2005). CASPubMedGoogle Scholar
- Davis, E., Chen, J., Leon, K., Darst, S. A. & Campbell, E. A. Mycobacterial RNA polymerase forms unstable open promoter complexes that are stabilized by CarD. Nucleic Acids Res.43, 433–445 (2015). CASPubMedGoogle Scholar
- Boyaci, H., Chen, J., Jansen, R., Darst, S. A. & Campbell, E. A. Structures of an RNA polymerase promoter melting intermediate elucidate DNA unwinding. Nature565, 382–385 (2019). First structural snapshot of an RNAP promoter melting intermediate. CASPubMedPubMed CentralGoogle Scholar
- Boyaci, H. et al. Fidaxomicin jams Mycobacterium tuberculosis RNA polymerase motions needed for initiation via RbpA contacts. eLife7, e34823 (2018). PubMedPubMed CentralGoogle Scholar
- Chen, J. et al. Stepwise promoter melting by bacterial RNA polymerase. Mol. Cell78, 275–288.e6 (2020). Captured structural snapshots of promoter melting intermediates that span the RNAP closed complex to the final transcriptionally competent open promoter complex. CASPubMedPubMed CentralGoogle Scholar
- McClure, W. R., Cech, C. L. & Johnston, D. E. A steady state assay for the RNA polymerase initiation reaction. J. Biol. Chem.253, 8941–8948 (1978). CASPubMedGoogle Scholar
- Carpousis, A. J. & Gralla, J. D. Cycling of ribonucleic acid polymerase to produce oligonucleotides during initiation in vitro at the lac UV5 promoter. Biochemistry19, 3245–3253 (1980). CASPubMedGoogle Scholar
- Goldman, S. R., Ebright, R. H. & Nickels, B. E. Direct detection of abortive RNA transcripts in vivo. Science324, 927 (2009). CASPubMedPubMed CentralGoogle Scholar
- Hsu, L. M., Vo, N. V., Kane, C. M. & Chamberlin, M. J. In vitro studies of transcript initiation by Escherichia coli RNA polymerase. 1. RNA chain initiation, abortive initiation, and promoter escape at three bacteriophage promoters. Biochemistry42, 3777–3786 (2003). CASPubMedGoogle Scholar
- Hsu, L. M. Monitoring abortive initiation. Methods47, 25–36 (2009). CASPubMedGoogle Scholar
- Kapanidis, A. N. et al. Initial transcription by RNA polymerase proceeds through a DNA-scrunching mechanism. Science314, 1144–1147 (2006). PubMedPubMed CentralGoogle Scholar
- Revyakin, A., Liu, C., Ebright, R. H. & Strick, T. R. Abortive initiation and productive initiation by RNA polymerase involve DNA scrunching. Science314, 1139–1143 (2006). Single-molecule studies revealing the role of scrunching in abortive initiation and promoter escape. CASPubMedPubMed CentralGoogle Scholar
- Winkelman, J. T. et al. Crosslink mapping at amino acid-base resolution reveals the path of scrunched DNA in initial transcribing complexes. Mol. Cell59, 768–780 (2015). CASPubMedPubMed CentralGoogle Scholar
- Duchi, D. et al. RNA polymerase pausing during initial transcription. Mol. Cell63, 939–950 (2016). CASPubMedPubMed CentralGoogle Scholar
- Kulbachinskiy, A. & Mustaev, A. Region 3.2 of the sigma subunit contributes to the binding of the 3’-initiating nucleotide in the RNA polymerase active center and facilitates promoter clearance during initiation. J. Biol. Chem.281, 18273–18276 (2006). CASPubMedGoogle Scholar
- Pupov, D., Kuzin, I., Bass, I. & Kulbachinskiy, A. Distinct functions of the RNA polymerase σ subunit region 3.2 in RNA priming and promoter escape. Nucleic Acids Res.42, 4494–4504 (2014). CASPubMedPubMed CentralGoogle Scholar
- Samanta, S. & Martin, C. T. Insights into the mechanism of initial transcription in Escherichia coli RNA polymerase. J. Biol. Chem.288, 31993–32003 (2013). CASPubMedPubMed CentralGoogle Scholar
- Li, L., Molodtsov, V., Lin, W., Ebright, R. H. & Zhang, Y. RNA extension drives a stepwise displacement of an initiation-factor structural module in initial transcription. Proc. Natl Acad. Sci. USA117, 5801–5809 (2020). CASPubMedPubMed CentralGoogle Scholar
- Dulin, D. et al. Pausing controls branching between productive and non-productive pathways during initial transcription in bacteria. Nat. Commun.9, 1478 (2018). PubMedPubMed CentralGoogle Scholar
- Jensen, D., Manzano, A. R., Rammohan, J., Stallings, C. L. & Galburt, E. A. CarD and RbpA modify the kinetics of initial transcription and slow promoter escape of the Mycobacterium tuberculosis RNA polymerase. Nucleic Acids Res.47, 6685–6698 (2019). CASPubMedPubMed CentralGoogle Scholar
- Mooney, R. A., Darst, S. A. & Landick, R. Sigma and RNA polymerase: an on-again, off-again relationship? Mol. Cell20, 335–345 (2005). CASPubMedGoogle Scholar
- Harden, T. T. et al. Bacterial RNA polymerase can retain σ70 throughout transcription. Proc. Natl Acad. Sci. USA113, 602–607 (2016). CASPubMedPubMed CentralGoogle Scholar
- Mukhopadhyay, J. et al. Translocation of σ70 with RNA polymerase during transcription: fluorescence resonance energy transfer assay for movement relative to DNA. Cell106, 453–463 (2001). CASPubMedGoogle Scholar
- Bar-Nahum, G. & Nudler, E. Isolation and characterization of sigma(70)-retaining transcription elongation complexes from Escherichia coli. Cell106, 443–451 (2001). CASPubMedGoogle Scholar
- Kapanidis, A. N. et al. Retention of transcription initiation factor sigma70 in transcription elongation: single-molecule analysis. Mol. Cell20, 347–356 (2005). CASPubMedGoogle Scholar
- Mooney, R. A. & Landick, R. Tethering sigma70 to RNA polymerase reveals high in vivo activity of sigma factors and sigma70-dependent pausing at promoter-distal locations. Genes Dev.17, 2839–2851 (2003). CASPubMedPubMed CentralGoogle Scholar
- Ring, B. Z., Yarnell, W. S. & Roberts, J. W. Function of E. coli RNA polymerase sigma factor sigma 70 in promoter-proximal pausing. Cell86, 485–493 (1996). CASPubMedGoogle Scholar
- Davis, M. C., Kesthely, C. A., Franklin, E. A. & MacLellan, S. R. The essential activities of the bacterial sigma factor. Can. J. Microbiol.63, 89–99 (2017). CASPubMedGoogle Scholar
- Gross, C. A. et al. The functional and regulatory roles of sigma factors in transcription. Cold Spring Harb. Symp. Quant. Biol.63, 141–155 (1998). CASPubMedGoogle Scholar
- Losick, R. & Pero, J. Cascades of sigma factors. Cell25, 582–584 (1981). CASPubMedGoogle Scholar
- Paget, M. Bacterial sigma factors and anti-sigma factors: structure, function and distribution. Biomolecules5, 1245–1265 (2015). CASPubMedPubMed CentralGoogle Scholar
- Staroń, A. et al. The third pillar of bacterial signal transduction: classification of the extracytoplasmic function (ECF) σ factor protein family: ECF σ factor classification. Mol. Microbiol.74, 557–581 (2009). A comparative genomic analysis revealing the range and diversity of the ECF σ-factor in bacteria. PubMedGoogle Scholar
- Hughes, K. T. & Mathee, K. The anti-sigma factors. Annu. Rev. Microbiol.52, 231–286 (1998). CASPubMedGoogle Scholar
- Campbell, E. A. et al. A conserved structural module regulates transcriptional responses to diverse stress signals in bacteria. Mol. Cell27, 793–805 (2007). CASPubMedPubMed CentralGoogle Scholar
- Maillard, A. P. et al. The crystal structure of the anti-σ factor CnrY in complex with the σ factor CnrH shows a new structural class of anti-σ factors targeting extracytoplasmic function σ factors. J. Mol. Biol.426, 2313–2327 (2014). CASPubMedGoogle Scholar
- Sineva, E., Savkina, M. & Ades, S. E. Themes and variations in gene regulation by extracytoplasmic function (ECF) sigma factors. Curr. Opin. Microbiol.36, 128–137 (2017). CASPubMedPubMed CentralGoogle Scholar
- Campbell, E. A. et al. Crystal structure of the Bacillus stearothermophilus anti-sigma factor SpoIIAB with the sporulation sigma factor sigmaF. Cell108, 795–807 (2002). CASPubMedGoogle Scholar
- Campbell, E. A. et al. Crystal structure of Escherichia coli sigmaE with the cytoplasmic domain of its anti-sigma RseA. Mol. Cell11, 1067–1078 (2003). CASPubMedGoogle Scholar
- Sorenson, M. K., Ray, S. S. & Darst, S. A. Crystal structure of the flagellar σ/anti-σ complex σ28/FlgM reveals an intact σ factor in an inactive conformation. Mol. Cell14, 127–138 (2004). CASPubMedGoogle Scholar
- Schumacher, M. A. et al. The crystal structure of the RsbN–σBldN complex from Streptomyces venezuelae defines a new structural class of anti-σ factor. Nucleic Acids Res.46, 7405–7417 (2018). CASPubMedPubMed CentralGoogle Scholar
- Gallagher, K. A. et al. c-di-GMP arms an anti-σ to control progression of multicellular differentiation in streptomyces. Mol. Cell77, 586–599.e6 (2020). CASPubMedPubMed CentralGoogle Scholar
- Shukla, J., Gupta, R., Thakur, K. G., Gokhale, R. & Gopal, B. Structural basis for the redox sensitivity of the Mycobacterium tuberculosis SigK-RskA σ-anti-σ complex. Acta Crystallogr. D Biol. Crystallogr.70, 1026–1036 (2014). CASPubMedGoogle Scholar
- Devkota, S. R., Kwon, E., Ha, S. C., Chang, H. W. & Kim, D. Y. Structural insights into the regulation of Bacillus subtilis SigW activity by anti-sigma RsiW. PLoS ONE12, e0174284 (2017). PubMedPubMed CentralGoogle Scholar
- Herrou, J., Rotskoff, G., Luo, Y., Roux, B. & Crosson, S. Structural basis of a protein partner switch that regulates the general stress response of α-proteobacteria. Proc. Natl Acad. Sci. USA109, 7973–7973 (2012). CASGoogle Scholar
- Campagne, S. et al. Structural basis for sigma factor mimicry in the general stress response of Alphaproteobacteria. Proc. Natl Acad. Sci. USA109, E1405–E1414 (2012). CASPubMedPubMed CentralGoogle Scholar
- Campbell, E. A., Westblade, L. F. & Darst, S. A. Regulation of bacterial RNA polymerase sigma factor activity: a structural perspective. Curr. Opin. Microbiol.11, 121–127 (2008). CASPubMedPubMed CentralGoogle Scholar
- Patikoglou, G. A. et al. Crystal structure of the Escherichia coli regulator of sigma70, Rsd, in complex with sigma70 domain 4. J. Mol. Biol.372, 649–659 (2007). CASPubMedPubMed CentralGoogle Scholar
- Shi, J. et al. Structural basis of σ appropriation. Nucleic Acids Res.47, 9423–9432 (2019). CASPubMedPubMed CentralGoogle Scholar
- Hu, Y., Morichaud, Z., Chen, S., Leonetti, J.-P. & Brodolin, K. Mycobacterium tuberculosis RbpA protein is a new type of transcriptional activator that stabilizes the σ A -containing RNA polymerase holoenzyme. Nucleic Acids Res.40, 6547–6557 (2012). CASPubMedPubMed CentralGoogle Scholar
- Bortoluzzi, A. et al. Mycobacterium tuberculosis RNA polymerase-binding protein A (RbpA) and its interactions with sigma factors. J. Biol. Chem.288, 14438–14450 (2013). CASPubMedPubMed CentralGoogle Scholar
- Tabib-Salazar, A. et al. The actinobacterial transcription factor RbpA binds to the principal sigma subunit of RNA polymerase. Nucleic Acids Res.41, 5679–5691 (2013). CASPubMedPubMed CentralGoogle Scholar
- Vishwakarma, R. K. et al. Single-molecule analysis reveals the mechanism of transcription activation in M. tuberculosis. Sci. Adv.4, eaao5498 (2018). PubMedPubMed CentralGoogle Scholar
- Hubin, E. A. et al. Structural, functional, and genetic analyses of the actinobacterial transcription factor RbpA. Proc. Natl Acad. Sci. USA112, 7171–7176 (2015). CASPubMedPubMed CentralGoogle Scholar
- Haakonsen, D. L., Yuan, A. H. & Laub, M. T. The bacterial cell cycle regulator GcrA is a σ70 cofactor that drives gene expression from a subset of methylated promoters. Genes Dev.29, 2272–2286 (2015). CASPubMedPubMed CentralGoogle Scholar
- Holtzendorff, J. et al. Oscillating global regulators control the genetic circuit driving a bacterial cell cycle. Science304, 983–987 (2004). CASPubMedGoogle Scholar
- Wu, X. et al. Structural insights into the unique mechanism of transcription activation by Caulobacter crescentus GcrA. Nucleic Acids Res.46, 3245–3256 (2018). CASPubMedPubMed CentralGoogle Scholar
- Gaal, T., Mandel, M. J., Silhavy, T. J. & Gourse, R. L. Crl facilitates RNA polymerase holoenzyme formation. J. Bacteriol.188, 7966–7970 (2006). CASPubMedPubMed CentralGoogle Scholar
- Banta, A. B. et al. Key features of σ S required for specific recognition by Crl, a transcription factor promoting assembly of RNA polymerase holoenzyme. Proc. Natl Acad. Sci. USA110, 15955–15960 (2013). CASPubMedPubMed CentralGoogle Scholar
- Cartagena, A. J. et al. Structural basis for transcription activation by Crl through tethering of σS and RNA polymerase. Proc. Natl Acad. Sci. USA116, 18923–18927 (2019). CASPubMedPubMed CentralGoogle Scholar
- Xu, J. et al. Crl activates transcription by stabilizing active conformation of the master stress transcription initiation factor. eLife8, e50928 (2019). CASPubMedPubMed CentralGoogle Scholar
- Wassarman, K. M. & Storz, G. 6S RNA regulates E. coli RNA polymerase activity. Cell101, 613–623 (2000). CASPubMedGoogle Scholar
- Trotochaud, A. E. & Wassarman, K. M. A highly conserved 6S RNA structure is required for regulation of transcription. Nat. Struct. Mol. Biol.12, 313–319 (2005). CASPubMedGoogle Scholar
- Barrick, J. E. 6S RNA is a widespread regulator of eubacterial RNA polymerase that resembles an open promoter. RNA11, 774–784 (2005). CASPubMedPubMed CentralGoogle Scholar
- Wassarman, K. M. in Regulating with RNA in Bacteria and Archaea Ch. 20 (eds Storz, G. & Papenfort, K.) 355–367 (ASM Press, 2019).
- Wassarman, K. M. & Saecker, R. M. Synthesis-mediated release of a small RNA inhibitor of RNA polymerase. Science314, 1601–1603 (2006). Discovery that 6S RNA serves as a functional template for transcription initiation by RNAP. CASPubMedGoogle Scholar
- Chen, J. et al. 6S RNA mimics B-form DNA to regulate Escherichia coli RNA polymerase. Mol. Cell68, 388–397.e6 (2017). CASPubMedPubMed CentralGoogle Scholar
- Lane, W. J. & Darst, S. A. Molecular evolution of multisubunit RNA polymerases: sequence analysis. J. Mol. Biol.395, 671–685 (2010). CASPubMedGoogle Scholar
- Lane, W. J. & Darst, S. A. Molecular evolution of multisubunit RNA polymerases: structural analysis. J. Mol. Biol.395, 686–704 (2010). CASPubMedGoogle Scholar
- Artsimovitch, I., Svetlov, V., Murakami, K. S. & Landick, R. Co-overexpression of Escherichia coli RNA polymerase subunits allows isolation and analysis of mutant enzymes lacking lineage-specific sequence insertions. J. Biol. Chem.278, 12344–12355 (2003). CASPubMedGoogle Scholar
- Chen, J. et al. E. coli TraR allosterically regulates transcription initiation by altering RNA polymerase conformation. eLife8, e49375 (2019). PubMedPubMed CentralGoogle Scholar
- Lin, W. et al. Structural basis of Mycobacterium tuberculosis transcription and transcription inhibition. Mol. Cell66, 169–179.e8 (2017). CASPubMedPubMed CentralGoogle Scholar
- Browning, D. F. & Busby, S. J. W. Local and global regulation of transcription initiation in bacteria. Nat. Rev. Microbiol.14, 638 (2016). CASPubMedGoogle Scholar
- Bergkessel, M. et al. The dormancy-specific regulator, SutA, is intrinsically disordered and modulates transcription initiation in Pseudomonas aeruginosa. Mol. Microbiol.112, 992–1009 (2019). CASPubMedPubMed CentralGoogle Scholar
- Molodtsov, V. et al. Allosteric effector ppGpp potentiates the inhibition of transcript initiation by DksA. Mol. Cell69, 828–839.e5 (2018). CASPubMedPubMed CentralGoogle Scholar
- Rutherford, S. T., Villers, C. L., Lee, J.-H., Ross, W. & Gourse, R. L. Allosteric control of Escherichia coli rRNA promoter complexes by DksA. Genes Dev.23, 236–248 (2009). CASPubMedPubMed CentralGoogle Scholar
- Gopalkrishnan, S., Ross, W., Chen, A. Y. & Gourse, R. L. TraR directly regulates transcription initiation by mimicking the combined effects of the global regulators DksA and ppGpp. Proc. Natl Acad. Sci. USA114, E5539–E5548 (2017). CASPubMedPubMed CentralGoogle Scholar
- Srivastava, D. B. et al. Structure and function of CarD, an essential mycobacterial transcription factor. Proc. Natl Acad. Sci. USA110, 12619–12624 (2013). CASPubMedPubMed CentralGoogle Scholar
- Bae, B. et al. CarD uses a minor groove wedge mechanism to stabilize the RNA polymerase open promoter complex. eLife4, e08505 (2015). PubMed CentralGoogle Scholar
- Venugopal, A. A. & Johnson, S. Fidaxomicin: a novel macrocyclic antibiotic approved for treatment of Clostridium difficile infection. Clin. Infect. Dis.54, 568–574 (2012). PubMedGoogle Scholar
- Srivastava, A. et al. New target for inhibition of bacterial RNA polymerase: ‘switch region’. Curr. Opin. Microbiol.14, 532–543 (2011). CASPubMedPubMed CentralGoogle Scholar
- Lin, W. et al. Structural basis of transcription inhibition by fidaxomicin (lipiarmycin A3). Mol. Cell70, 60–70.e16 (2018). CASPubMedPubMed CentralGoogle Scholar
- Belogurov, G. A. et al. Transcription inactivation through local refolding of the RNA polymerase structure. Nature457, 332–335 (2009). CASPubMedGoogle Scholar
- Mukhopadhyay, J. et al. The RNA polymerase “switch region” is a target for inhibitors. Cell135, 295–307 (2008). CASPubMedPubMed CentralGoogle Scholar
- Peek, J. et al. Rifamycin congeners kanglemycins are active against rifampicin-resistant bacteria via a distinct mechanism. Nat. Commun.9, 4147 (2018). PubMedPubMed CentralGoogle Scholar
- Mosaei, H. et al. Mode of action of kanglemycin A, an ansamycin natural product that is active against rifampicin-resistant Mycobacterium tuberculosis. Mol. Cell72, 263–274.e5 (2018). CASPubMedPubMed CentralGoogle Scholar
- Maffioli, S. I. et al. Antibacterial nucleoside-analog inhibitor of bacterial RNA polymerase. Cell169, 1240–1248.e23 (2017). CASPubMedPubMed CentralGoogle Scholar
- Zhang, Y. et al. GE23077 binds to the RNA polymerase ‘i’ and ‘i+1’ sites and prevents the binding of initiating nucleotides. eLife3, e02450 (2014). PubMedPubMed CentralGoogle Scholar
- McClure, W. R. On the mechanism of streptolydigin inhibition of Escherichia coli RNA polymerase. J. Biol. Chem.255, 1610–1616 (1980). CASPubMedGoogle Scholar
- Cassani, G., Burgess, R. R., Goodman, H. M. & Gold, L. Inhibition of RNA polymerase by streptolydigin. Nat. New Biol.230, 197–200 (1971). CASPubMedGoogle Scholar
- Siddhikol, C., Erbstoeszer, J. W. & Weisblum, B. Mode of action of streptolydigin. J. Bacteriol.99, 151–155 (1969). CASPubMedPubMed CentralGoogle Scholar
- Temiakov, D. et al. Structural basis of transcription inhibition by antibiotic streptolydigin. Mol. Cell19, 655–666 (2005). CASPubMedGoogle Scholar
- Tuske, S. et al. Inhibition of bacterial RNA polymerase by streptolydigin: stabilization of a straight-bridge-helix active-center conformation. Cell122, 541–552 (2005). CASPubMedPubMed CentralGoogle Scholar
- Artsimovitch, I., Chu, C., Lynch, A. S. & Landick, R. A new class of bacteria RNA polymerase inhibitor affects nucleotide addition. Science302, 650–654 (2003). CASPubMedGoogle Scholar
- Bae, B. et al. CBR antimicrobials inhibit RNA polymerase via at least two bridge-helix cap-mediated effects on nucleotide addition. Proc. Natl Acad. Sci. USA112, E4178 (2015). CASPubMedPubMed CentralGoogle Scholar
- Feng, Y. et al. Structural basis of transcription inhibition by CBR hydroxamidines and CBR pyrazoles. Structure23, 1470–1481 (2015). CASPubMedPubMed CentralGoogle Scholar
- Degen, D. et al. Transcription inhibition by the depsipeptide antibiotic salinamide A. eLife3, e02451 (2014). PubMedPubMed CentralGoogle Scholar
- McClure, W. R. & Cech, C. L. On the mechanism of rifampicin inhibition of RNA synthesis. J. Biol. Chem.253, 8949–8956 (1978). CASPubMedGoogle Scholar
- Campbell, E. a. E. A. et al. Structural mechanism for rifampicin inhibition of bacterial RNA polymerase. Cell104, 901–912 (2001). Structure of RNAP bound to the clinically used antibiotic rifampicin revealing the basis of inhibition. CASPubMedGoogle Scholar
- Campbell, E. A. et al. Structural, functional, and genetic analysis of sorangicin inhibition of bacterial RNA polymerase. EMBO J.24, 674–682 (2005). CASPubMedPubMed CentralGoogle Scholar
- Darst, S. A., Kubalek, E. W. & Kornberg, R. D. Three-dimensional structure of Escherichia coli RNA polymerase holoenzyme determined by electron crystallography. Nature340, 730–732 (1989). CASPubMedGoogle Scholar
- Gnatt, A. L., Cramer, P., Fu, J., Bushnell, D. A. & Kornberg, R. D. Structural basis of transcription: an RNA polymerase II elongation complex at 3.3 Å resolution. Science292, 1876–1882 (2001). CASPubMedGoogle Scholar
- Murakami, K. S. X-ray crystal structure of Escherichia coli RNA polymerase σ70 holoenzyme. J. Biol. Chem.288, 9126–9134 (2013). CASPubMedPubMed CentralGoogle Scholar
- Bae, B. et al. Phage T7 Gp2 inhibition of Escherichia coli RNA polymerase involves misappropriation of 70 domain 1.1. Proc. Natl Acad. Sci. USA110, 19772–19777 (2013). CASPubMedPubMed CentralGoogle Scholar
- Zuo, Y., Wang, Y. & Steitz, T. A. The mechanism of E. coli RNA polymerase regulation by ppGpp is suggested by the structure of their complex. Mol. Cell50, 430–436 (2013). CASPubMedPubMed CentralGoogle Scholar
- Plaschka, C. et al. Transcription initiation complex structures elucidate DNA opening. Nature533, 353–358 (2016). CASPubMedGoogle Scholar
- Kang, J. Y. et al. Structural basis of transcription arrest by coliphage HK022 Nun in an Escherichia coli RNA polymerase elongation complex. eLife6, e25478 (2017). PubMedPubMed CentralGoogle Scholar
- Liu, B., Hong, C., Huang, R. K., Yu, Z. & Steitz, T. A. Structural basis of bacterial transcription activation. Science358, 947–951 (2017). CASPubMedGoogle Scholar
- Stevens, A. An inhibitor of host sigma-stimulated core enzyme activity that purified with DNA-dependent RNA polymerase of E. coli following T4 phage infection. Biochem. Biophys. Res. Commun.54, 488–493 (1973). CASPubMedGoogle Scholar
- Wiggs, J. L., Gilman, M. Z. & Chamberlin, M. J. Heterogeneity of RNA polymerase in Bacillus subtilis: evidence for an additional sigma factor in vegetative cells. Proc. Natl Acad. Sci. USA78, 2762–2766 (1981). CASPubMedPubMed CentralGoogle Scholar
- Haldenwang, W. G. & Losick, R. A modified RNA polymerase transcribes a cloned gene under sporulation control in Bacillus subtilis. Nature282, 256–260 (1979). CASPubMedGoogle Scholar
- Buttner, M. J., Smith, A. M. & Bibb, M. J. At least three different RNA polymerase holoenzymes direct transcription of the agarase gene (dagA) of Streptomyces coelicolor A3(2). Cell52, 599–607 (1988). CASPubMedGoogle Scholar
- Erickson, J. W. & Gross, C. A. Identification of the sigma E subunit of Escherichia coli RNA polymerase: a second alternate sigma factor involved in high-temperature gene expression. Genes Dev.3, 1462–1471 (1989). CASPubMedGoogle Scholar
- Lonetto, M. A., Brown, K. L., Rudd, K. E. & Buttner, M. J. Analysis of the Streptomyces coelicolor sigE gene reveals the existence of a subfamily of eubacterial RNA polymerase σ factors involved in the regulation of extracytoplasmic functions. Proc. Natl Acad. Sci. USA91, 7573–7577 (1994). CASPubMedPubMed CentralGoogle Scholar
- Malhotra, A., Severinova, E. & Darst, S. A. Crystal structure of a σ70 subunit fragment from E. coli RNA polymerase. Cell87, 127–136 (1996). CASPubMedGoogle Scholar
- Rhodius, V. A. et al. Design of orthogonal genetic switches based on a crosstalk map of σs, anti-σs, and promoters. Mol. Syst. Biol.9, 702 (2013). CASPubMedPubMed CentralGoogle Scholar
- Campagne, S., Marsh, M. E., Capitani, G., Vorholt, J. A. & Allain, F. H. T. Structural basis for -10 promoter element melting by environmentally induced sigma factors. Nat. Struct. Mol. Biol.21, 269–276 (2014). CASPubMedGoogle Scholar
- Li, L., Fang, C., Zhuang, N., Wang, T. & Zhang, Y. Structural basis for transcription initiation by bacterial ECF σ factors. Nat. Commun.10, 1153 (2019). PubMedPubMed CentralGoogle Scholar
- Lin, W. et al. Structural basis of ECF-σ-factor-dependent transcription initiation. Nat. Commun.10, 710 (2019). CASPubMedPubMed CentralGoogle Scholar
- Li, S. et al. Structural basis for the recognition of MucA by MucB and AlgU in Pseudomonas aeruginosa. FEBS J.286, 4982–4994 (2019). CASPubMedGoogle Scholar
Acknowledgements
The authors thank R. Landick, S. Darst and R. Froom for helpful discussions and copyediting. They apologize to colleagues whose work could not be cited owing to the scope and space limits of the Review. The authors are grateful for support from NIH grant 2-R01 GM114450 (E.A.C.) and the Charles H. Revson Foundation award CEN5650030 (H.B.).